A new collection of Royal Society Biographical Memoirs celebrates the scientists who initiated the study of physiological mechanisms at the cellular level, uncovering the biophysical basis for how animal movement is initiated.
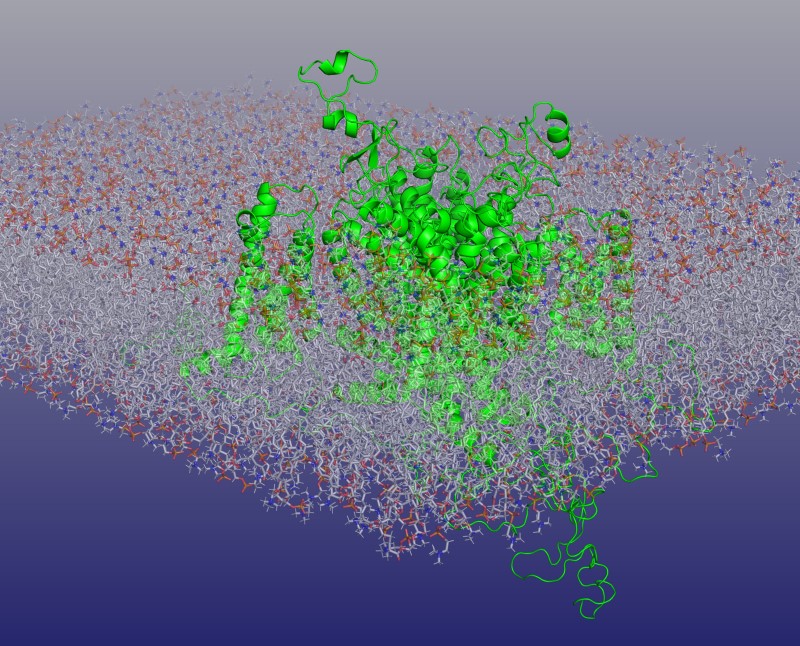
Initiation and execution of movement—whether voluntary action, the heartbeat or gastrointestinal motility—is fundamental to animal life. The underlying regulatory and executive structures have attracted much scientific study, in so doing, creating the field of Cell Physiology. Early pioneering studies—innovating techniques and instrumentation of ingenuity, elegance, and precision—investigated signal communication along and between nerve and muscle, leading to the advances represented in this collection.
A field with potential
In mid-to-late 19th Century Germany, Emil Du Bois-Reymond described bioelectric phenomena radiating from a stimulus site in living muscle; and Ludimar Hermann measured and defined its background electrical properties. Eduard Pflüger demonstrated that negative and positive polarities of electrical stimuli respectively triggered and terminated neuromuscular excitation; and Julius Bernstein measured and proposed the first hypothesis explaining the nerve impulse or action potential—the fundamental event associated with cell excitability.
From there, Keith Lucas demonstrated the ‘all or none’ nature of this key manifestation of excitation, and its transmission and temperature dependence. E.D. Adrian, Bryan Matthews and Herbert Gasser’s introduction of electrical recordings using string galvanometers, capillary electrometers and valve amplifiers, and their oscillographic display, permitted close analysis of the extremely small (mV-μV) electrical signals accompanying the nerve impulse. Thus began the electronic age of cell physiology and its application of physical concepts and techniques to the physiological sciences.
Recently invented glass microelectrodes demonstrated that these voltage changes took place across the cell membrane and the intracellular electrically relatively negative resting potential in muscle cells. Julius Bernstein attributed the latter to a Nernst potential across a selectively K+ permeable membrane separating solutions of high intracellular and low extracellular [K+]. Bernstein then attributed the positive-going nature of the action potential depolarization to this permeability becoming transiently nonselective. The membrane otherwise comprised insulating lipid bilaterally flanked by Helmholtz double layers and electrolyte. Thus Alan Hodgkin (related by marriage to Dorothy Hodgkin) and William Rushton demonstrated that resting nerves exhibited the direct current electrical properties resembling those previously demonstrated by Kelvin for an electrical cable.
Kenneth (Kacy) Cole (Fig. 1) initiated the era of modern biophysics studying squid giant axons, whose large diameters permitted intracellular, low resistance access by multiple electrodes. These made it possible to study for the first time membrane properties during the action potential whilst imposing alternating currents using a Wheatstone Bridge circuit (Fig. 1, right). These experiments associated action potentials with membrane conductance but not capacitance changes. Cole then invented the voltage clamp technique that could isolate membrane conductance and capacitance changes following imposed voltage steps.
Fig 1. Kacy Cole, acclaimed Father of modern biophysics, at the blackboard and his historic oscillograph record demonstrating Wheatstone bridge imbalances reflecting membrane conductance changes during the squid giant axon action potential (Cole & Curtis, 1939).
Former Royal Society Presidents, Alan Hodgkin and Andrew Huxley (related to writer Aldous Huxley) (Fig. 2), then separated the underlying inward and outward currents, characterizing their timecourses and voltage dependences. These were attributed, by Peter Baker and Trevor Shaw’s intracellular ionic replacement experiments, to Na+ and K+ currents respectively driven by the relatively high extracellular [Na+] and intracellular [K+]. Each current was modelled by transmembrane ohmic channel conductances gating with higher order voltage and kinetic dependences. Mathematically reconstructing their interactions with membrane voltage, Hodgkin and Huxley reproduced the observed regenerative depolarizing, recovery and refractory phases of the all-or-nothing action potential and its propagation. These excitation events superimposed upon the background resting potential explicable by Nernst-Planck analysis of the underlying electrodiffusive processes. Then followed Murdoch Ritchie and Huxley’s pharmacological channel quantifications and localization, clarification of the physical and anatomical determinants of conduction velocity, and its in vivo enhancement by nerve fibre myelination.
Fig 2. Huxley (left) and Hodgkin posing on the cover of a Nobel Prize booklet. They shared the 1963 prize in Physiology or Medicine with John Eccles.
Active membrane transport
Having clarified the immediate electrophysiological events underlying the action potential there remained the question of maintaining its driving intra- and extracellular ion gradients to allow repeated signalling along the neuron.
Hodgkin and Richard Keynes’s (great-grandson of Charles Darwin) radioactive tracer experiments showed that energetically driven, active, Na+/K+-ATPase transport—first demonstrated by Hans Ussing and Jens Skou and later further explored by David Gadsby—could establish, maintain or restore these essential transmembrane ion gradients. The phosphate metabolism energetically driving such transport activity was then investigated by Peter Baker, Trevor Shaw and Peter Caldwell. Such active processes are additionally fundamental in maintaining the integrity of all living cells against background osmotic destabilization by their intracellular charged protein, first described by George Donnan. They were integral to August Krogh’s earlier suggestion of “large differences in concentration of individual ions between the interior of living cells and the fluids surrounding them … brought about and also maintained by some special activity on the part of the cell, while diffusion processes are all the time tending to reduce them” and his concept of “… steady states, differing… from equilibria by the necessity of energy being supplied for their maintenance”. All these concepts were later quantitatively unified by relating the physical factors determining cell volume to those generating the cell resting potential. This involved an application of Gauss’s flux theorem to their intracellular charge differences.
Cell-to-cell
Membrane activation next propagates along the nerve to presynaptic terminals, functionally contacting and activating postsynaptic muscle fibres. Bernard Katz (Fig. 3), Paul Fatt and Ricardo Miledi, electrophysiologically demonstrated probabilistic, positive-going, 1-2 mV, miniature endplate potentials localized to subsynaptic membrane in resting muscle. These signalled individual quantal events, each releasing discrete quantities of the presynaptic transmitter acetylcholine. The latter would then diffuse and bind to specific postsynaptic transmitter- (as opposed to voltage-) activated ion channels. This would increase both Na+ and K+ permeation causing the momentary depolarizations. Numerous, synchronized events produced by presynaptic stimulation would sum to larger endplate potentials. If of sufficient amplitude, the latter would trigger postsynaptic Na+ channels, completing the cell-to-cell excitation process.
Paul Fatt and John Eccles extended these ideas to central nervous system synaptic activation between nerve cells. Additional to excitatory, they demonstrated inhibitory, postsynaptic potentials reflecting Cl- conductances. Graded summation of excitatory and inhibitory potentials to trigger action potentials furnished a basis for neuronal computation. Finally, the variety of biological transmitter species was broadened by Otto Loewi, Henry Dale, Ulf von Euler and Julius Axelrod.
Fig 3. Bernard Katz in his University College London (UCL) lab. Katz is one example of refugee Fellows who left mainland Europe leading up to World War II.
Action
Membrane activation then initiates the activity specific to the target cell. Here, Setsuro Ebashi implicated intracellular Ca2+ as a widespread initiating messenger. At the presynaptic terminal, Peter Baker and Trevor Shaw explored triggering of exocytotic transmitter release by intracellular [Ca2+] elevation within the nerve terminal.
Following synaptic activation, muscle membrane similarly initiates propagating action potentials. Richard Adrian (in the footsteps of his father, E.D. Adrian) demonstrated that these also involved voltage-dependent ion channel activity, extending such mechanisms to excitable cells generally. The excitation-contraction coupling that follows culminates in a Ca2+-mediated activation of contractile organelles in the cell interior. However, A.V. Hill had pointed out that reaching these from the cell surface would involve diffusion distances incompatible with the observed activation timescales. Instead, a muscle interior sarcoplasmic reticular store performs a local Ca2+ release through intracellular membrane ryanodine receptor channels. This release is activated by electrical signals conveyed from the surface by the invaginating transverse tubular membrane system described by George Palade. Thus Paul Fatt’s impedance analysis studies demonstrated that these could transmit surface electrical excitation.
Richard Adrian then reconstructed these requisite tubular voltage changes activating muscle contraction, and demonstrated the voltage-sensing processes through which these would trigger the release of store Ca2+. The latter were detected through the charge movements they produced with voltage change (Fig. 4). Extensive pharmacological and dielectric analyses identified the voltage sensors as modified tubular membrane Ca2+ channels coupled to the ryanodine receptor channels, and elucidated their operating mechanisms from their dielectric characteristics. Finally, recent work has suggested regulatory feedback loop actions of the released Ca2+ on the originating impulse production itself.
Fig 4. Electrophysiological demonstration of membrane molecular events: voltage detection events triggering activation of muscle contraction. Charge movements elicited by varying voltage steps produced by the tubular voltage sensor.
Legacy
From the outset, these lines of enquiry prompted explorations of other important biological systems. Sylvio Weidmann’s pioneering cardiac experiments culminated in our current understanding of cardiomyocyte physiology, its involvement in cardiac arrhythmias and their clinical management; Edith Bulbring established smooth muscle as an important area for investigation, laying foundations for our understanding of its function and autonomic regulation; and Michael Berridge’s explorations of Ca2+ mediated triggering extended to further cell types. These directions were aided by technical developments exemplified by Roger Tsien’s spectrofluometric optical dyes and William Catterall’s (Memoir in preparation) biochemical characterizations of the ion channels—themselves exemplified by the perspective cryogenic electronmicroscopic cardiac Na+ channel image featured (top figure).
Gasser, Adrian, Hill, Hodgkin, Huxley, Eccles, Krogh, Katz, Loewi, Dale, Palade, von Euler, Axelrod, Skou, Neher, Sakmann and Tsien won Nobel Prizes in Medicine or Physiology, or Chemistry. These accolades affirm Cell Physiology’s rigorous and productive legacy that will continue to generate future avenues for physiological inquiry and its translation into medical practice.
All of the memoirs discussed here are available to read in the new Cell Physiology collection. Biographical Memoirs are published online as they are ready before being compiled into two volumes per year. Sign up for alerts or keep an eye on our website for new memoirs as they appear.
Image credits:
Top image: Na+ channel portrayed by computational reconstruction modelling of the cardiac Nav1.5 cryo-electronmicroscope structure within lipid bilayer membrane. Adapted from cover illustration, Phil Trans R Soc (2023). Volume 378, Issue 1879 Kindly provided by Christopher Beaudoin and Antony P Jackson, Department of Biochemistry, University of Cambridge, UK.
Fig 1. A) Kenneth (Kacy) Cole, copyright unknown. B) Fig. 4 from Electric Impedance Of The Squid Giant Axon During Activity. Cole & Curtis. J Gen Physiol (1939) 22 (5): 649–670, used with permission.
Fig 2. Nobel Prize booklet cover 1963, copyright unknown.
Fig 3. Sir Bernard Katz in the UCL lab. From UCL Special Collections, copyright unknown.
Fig 4.) Fig. 2A from Huang CLH (1994) Charge conservation in intact frog skeletal muscle fibres in gluconate-containing solutions. J Physiol. 1994 Jan 1;474(1):161-71, provided by the author.